Arabidopsis Response Regulator 6 (ARR6) Modulates Plant Cell-Wall Composition and Disease Resistance
Texte intégral
Figure
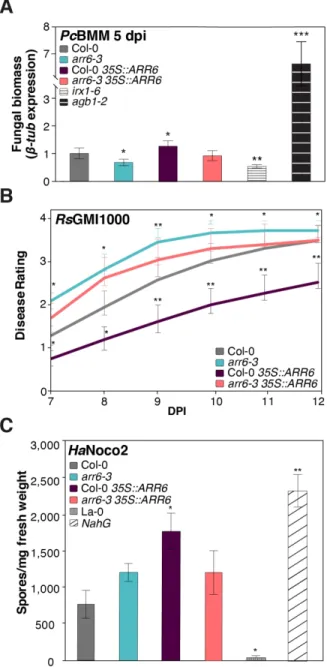

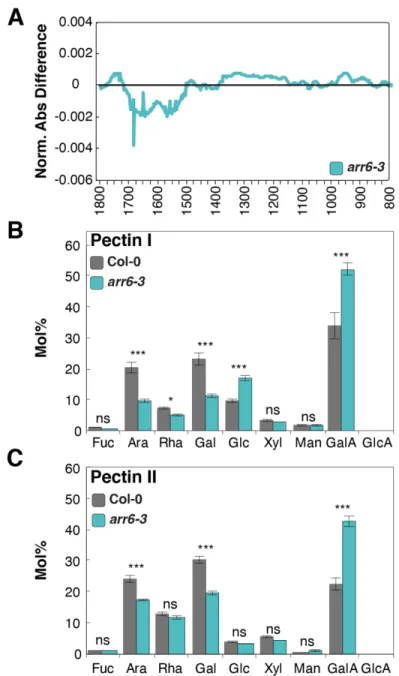

Documents relatifs
We conclude that plant crude extracts containing H5 oligomers are more immunogenic than the trim- eric H5-S·Tag containing extracts as measured by an Figure 7 Strong and
Results showed a well defined localization of cell wall epitopes with highly esterified homogalacturonan and arabinogalactan-protein mainly in the tip region, weakly
Accordingly, after 10 days of drought, significant changes in a number of secondary metabolites for the mutant lines trxo1-1 and trxo1-2 were observed, including
[r]
Values are expression levels relative to the water control (defined value of 1). Experiments were repeated twice with similar results... further tested whether BABA-IR is functional
triple mutant exhibited a higher callose deposition than sid2 plants... We also studied callose accumulation in leaf tissues in response to mechanical
(C) Root lengths of Col-0 and azi1 seedlings grown with Azospiril- lum brasilense (Sp245) on +Zn or –Zn medium for five days. Data are represented as mean ± s.d.. Letters
By contrast to the idd4 mutant, the overexpression of IDD4 (IDD4ox) reduces defense- related gene expression depicted by the GO analysis of significantly down-regulated