Accurate reactivity effects of pwr control rods using apollo3 advanced solvers
Texte intégral
Figure


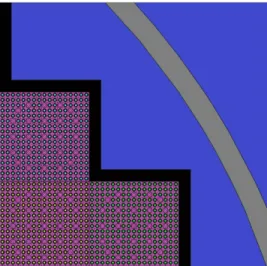

Documents relatifs
En 2005, un peu plus de huit candidats sur dix visant un diplôme de niveau V se présentaient à des certifications dans le domaine sanitaire et social (tableau 1) : DPAS
Ten non-disabled subjects performed a reaching task, while wearing an elbow prosthesis which was driven by several interjoint coordination models obtained through different
Nous pouvons reconnaître que choisir 2 boules simultanément d’une urne de 4 boules est une appli- cation injective où l’ordre n’est pas important.. Donc l’ordre aura
À cet égard, les soins du développement constituent un regroupement d’interventions de soins qui visent à favoriser le développement du prématuré hospitalisé à
Abstract: This study reports the removal of amoxicillin (AMX) in aqueous media using the electro-Fenton process in the presence of a graphite cathode recovered from used
The first one is used to prove just one property, the second to prove a list of mutually dependent properties(like.. the proof of periodicity in the example below). Inputs of
This experiment allowed us to quantify order and disorder in a configuration, using a geometrical measure of stacking and a mechanical measure of effective suscep- tibility,
beginning of the polymerization; linear growth with conversion; lower polydispersities), additional experiments were carried out with this compound at higher temperatures